Designing Better Batteries
Lithium-ion batteries revolutionised portable electronic devices, making them smaller and lighter than ever before. But they’ve hit their limit. More powerful and lightweight batteries with even higher energy densities will be required to further miniaturise devices, or to power larger vehicles like trucks and aeroplanes.
Prof. Michelle Spencer, from Applied Chemistry and Environmental Science at RMIT University, is using computer modelling to understand exactly what happens between the anode and cathode of next-generation lithium-metal batteries, in search of the best materials to ensure safe and reliable operation of these powerful batteries.
The Challenge
Despite the widespread adoption of lithium-ion battery technology, limitations in their energy and power density still prevent their use in many advanced devices and vehicles. Efforts to develop next-generation rechargeable battery technologies are still based on lithium-ion as the moving charge, but use a lithium metal anode rather than a graphite anode. These lithium metal batteries can provide a 10-fold increase in energy density, potentially unlocking high-powered battery applications from transport to miniaturised electronics.
Unfortunately, the conventional organic solvent-based electrolytes used in lithium-ion batteries are flammable and reactive with lithium metal, causing continuous reactions that compromise both safety and long-term battery performance.
Ionic liquids have been proposed as new electrolytes as they are inherently safe (very low flammability), have high conductivity, and good thermal and chemical stability. Prof. Spencer explains: “It is essential that the repetitive deposition and stripping of lithium ions at the lithium metal surface, which is the charge movement that occurs during normal battery operation, must occur without significant reaction or decomposition of the electrolyte in contact with it. So, the goal is to design an ionic liquid that will form a stable passive layer at the lithium metal surface but still allow lithium ions through.”
Several different ionic liquid electrolytes have demonstrated stable battery performance, but it is not known why some perform better than others. “They are likely to form a solid electrolyte interphase (SEI) layer on the lithium metal surface,” says Prof. Spencer, “but the complex composition and structure of these SEIs are very difficult to characterise experimentally – they are thin films only nanometres thick. This makes designing the best electrolyte difficult.”
The Solution
Prof. Spencer and her colleagues Dr Michael Breedon at CSIRO Manufacturing and Dr Thomas Rüther at CSIRO Energy are using a combination of experimental testing, physical characterisation and molecular modelling to understand the formation and structure of effective SEI layers. But with COVID-19 limiting access to physical laboratories, computational experiments using Pawsey’s supercomputing facilities have allowed the research to progress.
The team used density functional theory calculations and ab initio molecular dynamics simulations to determine how the various components of ionic liquid electrolytes preferentially adsorb and then react on a lithium metal surface. They have also examined the properties of the lithium salt that forms part of the electrolyte mixture. “Using these simulations, we could better understand the stability and electronic properties of the electrolyte, the most likely reactions occurring at the anode surface, identify any decomposition products, and determine how the resulting SEI layer can stabilise the lithium anode,” says Prof. Spencer. “If no gaseous (expanding) products form and the SEI is stable and inert after that initial contact and surface reaction, it’s likely to enable safer cycling of the battery. Our calculations can also provide estimates of the ionic conductivity and the oxidation and reduction potential of the electrolyte components, which can determine their effective working voltage range in a battery.”
Outcomes
“Although we’re only modelling 100-200 atoms at a time, the calculations are very computationally intensive, as we’re making and breaking chemical bonds – if I was running this on my desktop computer, it would still be calculating long after I’d retired. But with Pawsey facilities we’ve been able to perform tens of thousands of calculations to study hundreds of electrolyte components and their many complex interactions and reactions with the lithium metal surface at a range of operating temperatures.”
Prof. Spencer’s modelling work has confirmed that a new class of ionic liquids based on boronium cations are viable candidates for rechargeable lithium metal batteries, showing high electrochemical and thermal stability as well as forming a relatively inert SEI layer. Insights gained into their SEI formation and structure at a molecular level are now being used to guide the experimental development of safe, long-life, high-capacity rechargeable lithium metal batteries that could power the transport of the future.
Project Leader.
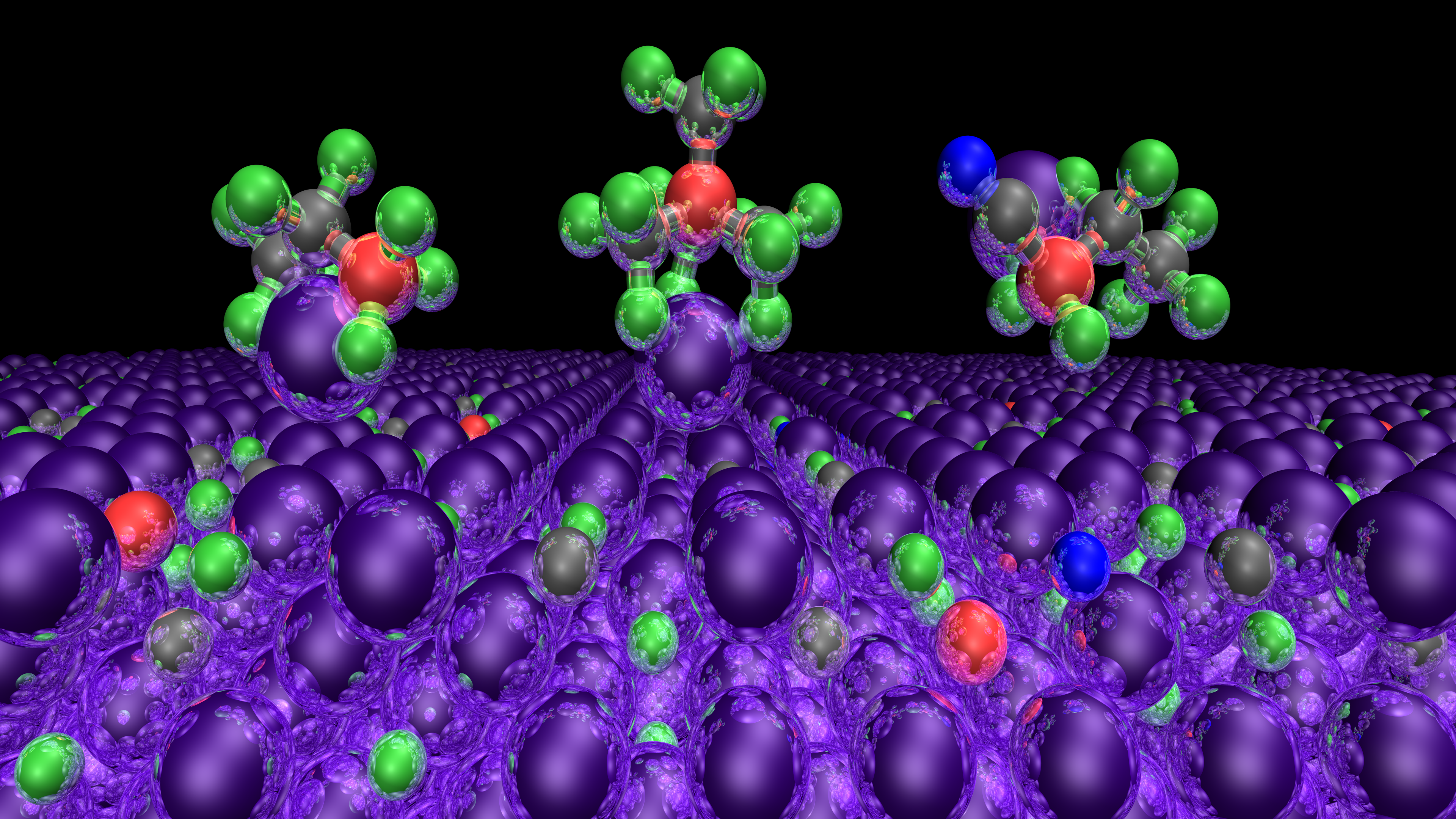
AIMD simulation of the reaction between borate anion electrolyte molecules with a Li(001) anode surface. (Image created by Dale Osborne and Jonathan Clarke-Hannaford)